High-Resolution Cell Subset Enumeration by Mass Cytometry
Cells are the quanta of the immune system, and their identity and function can be understood by the degree to which they express proteins on the cell surface or intracellularly. Traditionally, the work-horse tool of immunology has been the flow cytometer, which optically measures the return fluorescence from cells stained with fluorophore-labeled antibodies bound to proteins. It is through functional characterization of cells expressing specific combinations of proteins that much of our understanding of immune system functionality has been gained and the many different cell types, each with their own functionality, have been delineated. Thus, a drive towards being able to measure an increasing number of fluorophores on single cells was present from the early days of flow cytometry development.
1 Yet a difficulty for using multiple fluorophores simultaneously in flow cytometry is that the fluorescence emission spectrum of one fluorophore “spills over” from its characteristic wave length to interfere with readings from other fluorophores. This strongly reduces the ability to accurately gauge the abundance of each protein in the cell. Thus, at expert flow cytometry centers, with substantial effort one can use up to 10–15 different fluorophores before the overlapping emission spectra become too complex to be accurately separated, whereas for most flow cytometry centers, let alone clinically based ones, it would be a struggle to accurately measure even that many labels together.
Mass cytometry is a recently introduced technology (the commercial product is called a “CyTOF” for cytometry by time-of-flight; DVS Sciences, Mountain View, CA, USA) that measures the abundance of heavy metal isotope labels on antibodies and other tags (such as peptide-MHC tetramers for labeling specific T cells) on single cells using mass spectroscopy.2,3 Unlike flow cytometry, which is grounded in the physics of optics, the advantage of mass cytometry is that no optical equivalent of “spill-over” exists in mass, such that many more molecules may be used in combination to assay a single sample (blood or single cell suspension of tissues).4–7 Currently, isotopic-label antibody panels spanning over 35 proteins are already run regularly via mass cytometry (110 different proteins is the upper limit), and much more information may be obtained from each cell. An illustration of the revolution in scale this transformative technology provides is that while 10 labels may yield approximately 1,000 possible combinations of cell subsets, 30 yield 1,000,000 (using the formula X = 2n−1, where X is the number of combinations and n is the number of labels). Of note, repeated runs of a flow cytometer on the same sample, each time measuring a different combination of antibodies, will not have equivalent coverage to the mass cytometer as the data will not be obtained on the same single cell. Thus there is much greater resolving power with the number of labels possible with this new technology, enabling simultaneous quantification of the majority of known immune cell types from an individual sample, including rare cell subsets (Figure 2).
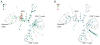 | Figure 2 Mass cytometry captures a snapshot of immune system alterations in cell subset abundance between conditions. |
Functional Signaling Assays at the Single Cell Level
For primary tissue, original applications of flow cytometry allowed only the quantification of cell surface markers. However, developments over the past decade, primarily in the lab of Garry Nolan at Stanford University, have enabled the quantification of intracellular proteins, and of particular interest, phosphorylated epitopes.
8,9 This allows for accurate quantification at the single cell level of phospho-signaling biochemistry (phospho-flow). Performed comparatively for a sample between an unstimulated and stimulated condition, the resultant difference or fold-change is interpreted as the degree of activation of the specific signaling pathways probed.
9,10 With mass cytometry, the strength of this approach has grown even further as it allows multi-parameterization at an even greater scale including both cell type and functional response to stimulation by cell surface intracellular markers respectively.
4,5,11,12 Thus, the machinery and systems now exist to access the heterogeneity of cellular subsets in the immune system and enable phenotypic characterization and functional assays at a resolution previously unavailable to immunologists.
Multiplex Measures of Serum Proteins
The immune system is very much dependent on the interactions of various white blood cells with each other, either in synaptic contacts, or at a distance using secreted proteins such as cytokines or chemokines for regulation and response. Cytokine levels in body fluids, such as serum or cerebrospinal fluid, can be quantitated, and due to their importance for immunity their measurement has always been of interest. Yet, the family of cytokines, chemokines, and more generally secreted factors involved in immunity is large, including many redundancies in its effects on cellular response and regulation, with many cytokines exhibiting multiple functionality that is context-dependent. Until recently only a handful of cytokines could be measured simultaneously in a study, and the resultant partial capture of this complex milieu did not yield high clinical utility.
In recent years, several technological platforms enabling simultaneous measurement of serum protein in multiplex have become available. Most popular amongst them are the bead array multiplex assays based on the Luminex technology (Luminex Corporation, Austin, TX, USA). These can measure up to 500 analytes from very small blood volumes, for almost 100 samples at a time. Kits geared for immune-phenotyping of up to 51 serum proteins in one assay well are already available from several vendors. Hence, it is a new day for attempts to identify predictive signatures of disease associations from body fluid-detectable proteins.
TCR and BCR Repertoire Analysis through Next-Generation Sequencing
The adaptive arm of immunity tailors responses for any encountered antigen. To do so, B and T cells generate an enormous repertoire of structural diversity in antigen-recognizing proteins, including antibodies and T cell receptors (TCR), through a gene segment rearrangement process which combines variable, diverse, and joining gene segments, known as VDJ recombination. An allelic exclusion mechanism generally allows only a single VDJ combination to be expressed in a given cell, despite the additional chromosomal copies, and a separate mechanism-activated post-antigen recognition assures high specificity of the receptor/antibody to the antigen through hyper-mutation and selection. As many as 10
8 different combinations can be created by VDJ recombination, and repertoire diversity is thought to be critical for protective immunity. With an estimated cell count of 10
11 different B and T cells in an individual human being, it is assumed that this mechanism generates a sufficiently large repertoire for immune system antigen recognition. However, until recently, surveying even a small fraction of an individual’s repertoire was considered an impossible task.
Next-generation DNA sequencing now offers the opportunity of starting to explore the basic principles of repertoire selection as well as its relation to disease. Through the design of primers flanking regions of interest, in-depth sequencing of a representative sampling of repertoire diversity may be achieved. First studies performing deep sequencing of antibody and TCR sequences have all reported that the VDJ recombination is biased.13–15 That is, it does not occur with equal probability for each combination. Moreover, large numbers of distinct combinations are expressed in a single individual at a single point in time15 (over 106 for TCR in humans, though still negligible compared with the upper theoretical limit of 1011), and a significant overlap of sequence repertoires between any two individuals can be observed.16 Similarly, a high-bandwidth low-cost deep sequencing approach has recently been described for sequencing human leukocyte antigen (HLA) regions.17 The size, diversity, and affinity of this repertoire is expected to be closely linked with immune response. Hence, exploring this diversity and its clinical implications in an individual or a population is of high importance.
Cell Type-Specific and Single Cell Gene Expression Assays
Much of our knowledge in immunology comes from bulk measurements of many cells together. Due to problems of averaging and noise, the behavior of cells as inferred from average measurements often drowns cell-to-cell differences and may not reflect the behavior of any single cell.
18 Beyond measuring a select few biological species across many single cells and different cell types (e.g. in flow or mass-based cytometry), measurement of many biological species (e.g. whole genome gene expression) has been dictated by cost limitations, technological hurdles, and the difficulty of analyzing
en masse single cell data. Hence, it is often the case that multiple cell types are analyzed as a single tissue, such as happens for example when gene expression is analyzed in bulk from whole blood, and the resulting analysis to a large degree describes the heterogeneity of the tissue, rather than the underlying biological changes in condition that are of interest.
Recent methodological and technological innovations now elevate some of these difficulties and enable the in-depth exploration of several biological species in many cell types or single cells. These include computational methodologies to infer cell type-specific information from heterogeneous tissue data,19,20 microfluidic devices used to measure and image cells run in multiplex (across multiple cells and genes),21,22 and, emerging now, single cell whole genome measures.23,24 These methodologies and technological innovations have enabled the measurement of single cell cytokine secretion25 and cell counting from minute samples,26,27 to name but a few. The miniaturization of these devices and their relative low cost and transportability are promising for the future development of microfluidics-based diagnostics. The sensitivity of cell type-specific measures performed through these techniques often offers orders of magnitude higher resolution than that obtained by analyzing heterogeneous measures of tissue and cells and reveal novel biological phenomena masked by cell-to-cell or cell type-to-cell type variation.