Anthracyclines are one of the most widely prescribed and effective cytotoxic drugs, used in the treatment of cancer. Chemotherapy regimens that include anthracycline are vastly used in the management of hematological and solid tumors, including breast cancer, lymphoma, leukemia, and sarcomas.10,11 Anthracycline-based regimens are used in approximately 1,000,000 patients annually in North America.12 Anthracycline-induced cardiotoxicity is the most well-studied chemotherapy-induced cardiovascular toxicity, first described in 1971.13
Mechanisms of Anthracycline-induced Cardiotoxicity
The classical hypothesis for the mechanism of anthracycline-induced cardiomyopathy entails generation of excess free radicals during doxorubicin metabolism. The quinone-hydroquinone moiety of anthracyclines undergoes reduction by oxidoreductases to a doxorubicin-semiquinone radical or doxorubicinol.
14 This quickly regenerates the parent quinone by reducing molecular oxygen to superoxide anion (O
2−), and dismutation of the latter generates hydrogen peroxide (H
2O
2).
15 In the presence of iron, Fe
3+–anthracycline complexes are formed, which further catalyze the conversion of H
2O
2 to other reactive oxygen species (ROS), including toxic hydroxyl radicals (OH
−; Fenton’s reaction). Conditions leading to higher iron tissue concentrations such as heterozygosity for C282Y, the hereditary hemochromatosis gene, may favor the development of doxorubicin cardiotoxicity.
16
The cardiac mitochondria are key intracellular targets for anthracyclines, both as sites of generation of free radical intermediates, and the mitochondrial-localized production of ROS can induce mitochondrial DNA mutation and disruption of bioenergetics.17,18 Reactive oxygen species-producing enzymes such as NAD(P)H oxidase are localized in the mitochondria.
Doxorubicin (DOX) alters iron trafficking inside the cell by increasing iron incorporation into ferritin and suppressing iron release from cellular stores.19 Furthermore, doxorubicin leads to preferential accumulation of iron in the mitochondria, by suppressing expression of ABC protein-B8 transporter, functioning in iron export out of the mitochondria.20
Doxorubicin exerts its tumoricidal activity via binding to topoisomerase-IIα (Top2α),21 an enzyme found predominantly in dividing cells and required for DNA replication. Doxorubicin binds both DNA and Top2 to form the ternary Top2-doxorubicin-DNA cleavage complex, with subsequent blockage of DNA resealing during cell replication.
More recently, Top2β (the isoform expressed in quiescent cells including cardiomyocytes) has been identified as a molecular mediator of DOX cardiotoxicity.22 Top2 inhibition by DOX causes double-stranded breaks in DNA and activation of DNA damage response pathways involving p53-mediated apoptosis. Activation of p53 is followed by repression of genes involved in mitochondrial biogenesis and oxidative phosphorylation pathways. Mice with a cardiac-specific deletion of Top2β are protected from doxorubicin-induced cardiotoxicity.22
Clinical Presentation
Cardiotoxicity may appear as asymptomatic or symptomatic reductions of left ventricular ejection fraction (LVEF) and may be acute or chronic.
23 Anthracycline cardiotoxicity has several distinct presentations including acute, early-onset, and late-onset dependent on the time of exposure.
24
Acute toxicity is uncommon (about 1%), and resembles an acute toxic myocarditis manifesting as transient left ventricular (LV) dysfunction, electrocardiographic changes, and arrhythmias, and develops immediately after a single dose, or a single course. Acute toxicity is believed to be reversible, and generally does not portend future development of heart failure.3,24–26 However, acute cardiac dysfunction may also reflect myocyte injury that eventually can evolve into early or late cardiotoxicity.3
Early-onset toxicity manifests within 1 year of anthracycline exposure23 and can lead to dilated cardiomyopathy. By definition, late-onset toxicity occurs more than 1 year after completion of therapy,3 presenting as progressive dilated cardiomyopathy. Its true incidence is not known, and it may represent post-anthracycline cardiac vulnerability to stressors and cardiovascular risk factors.27 The distinction between early- and late-onset is arbitrary and has recently been challenged. Cardinale et al. have shown that anthracycline cardiotoxicity occurs almost exclusively within the first year after completing treatment (98% of cases developed in the first year, with 3.5 months’ median time from the final dose of anthracycline to cardiotoxicity).6 These results suggest that late-onset anthracycline cardiotoxicity may reflect, at least in part, late diagnosis of early untreated cardiotoxicity.
Risk Factors and Risk Assessment
Several treatment- and patient-related factors determine the risk for anthracycline cardiotoxicity (
Table 1). Cumulative dose is the most important predictor of myocardial injury. Initial studies observed an exponential increase in the incidence of heart failure after a cumulative doxorubicin dose (5%, 26%, and 48% at cumulative doses of 400, 550, and 700 mg/m
2, respectively).
23 Subsequent studies suggested a lower threshold for cardiac dysfunction, with 7%, 9%, 18%, 38%, and 65% at cumulative doses of 150, 250, 350, 450, and 550 mg/m
2, respectively.
32 Other more recent studies support a cutoff of doxorubicin ≥250 mg/m
2 or equivalent to define the high-exposure category.
28,33,34 Table 2 depicts the maximal recommended dose for various anthracyclines.
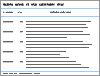 | Table 1Risk Factors for Anthracycline Cardiotoxicity. 5,28–31 |
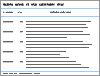 | Table 2Anthracycline Toxicity Equivalence Ratios. 35 |
Patients are considered at high risk for anthracycline cardiotoxicity if receiving high-dose treatment (e.g. doxorubicin ≥250 mg/m2). Patients receiving lower-dose anthracycline (e.g. doxorubicin <250 mg/m2) are also considered at high risk if they have any of the risk factors in Table 1.29 From a clinical perspective, one may assume that any previous myocardial insult can potentially make the patient more susceptible to anthracycline-induced cardiotoxicity.5
Lower-dose anthracycline therapy (e.g. doxorubicin <250 mg/m2) with no other risk factors is usually well tolerated.5 However, a sizable individual variation exists in the susceptibility to anthracycline cardiotoxicity, and even low cumulative doses may modestly increase the risk for cardiac cardiotoxicity29 and may be associated with subclinical LV dysfunction.37
Cardiac Imaging in Patients Undergoing Cancer Therapy
Routine surveillance imaging is recommended in asymptomatic patients considered to be at increased risk of developing cardiac dysfunction.
29 Echocardiography is the cornerstone in the evaluation of patients prior, during, and after anthracycline therapy. However, echocardiography has low sensitivity for the detection of small reductions in LV function and reliably detects differences close to 10% in LVEF.
38
Sub-clinical cardiotoxicity is commonly defined on cardiac imaging as a reduction in LVEF by >10% points to a value of EF <50% using echocardiography or equilibrium radionuclide angiography (MUGA).6,39,40 This definition has been endorsed by the American Society of Echocardiography and the European Association of Cardiovascular Imaging with a slight modification: LVEF decrease of >10% from baseline to a value <53%. This cutoff was chosen because data from six databases indicate that LVEF in the range of 53% to 73% should be classified as normal. The decrease in LVEF should be confirmed by repeated cardiac imaging, performed 2 to 3 weeks after the baseline diagnostic study showing the initial decrease in LVEF.38 This definition applies to all cancer therapeutics-related cardiac dysfunction (CTRCD).38
Global longitudinal strain (GLS) has been used to detect subtle alterations in systolic function, in an attempt to predict subsequent drops in LVEF.39,41 Reduction of GLS >15% from baseline occurring prior to any change in LVEF has been shown to precede the decrease in LVEF and can be used for the detection of subclinical LV dysfunction.38 The ANMCO/AIOM/AICO Consensus Document on clinical and management pathways of cardio-oncology accepts a GLS drop >10% as an indicator of subclinical LV dysfunction that warrants consideration of cardioprotection.42 However, there have been no studies to demonstrate that early intervention based on change in strain alone (in the absence of a reduction in LVEF) predicts the development of clinical heart failure or translates to a reduction in symptomatic cardiac dysfunction. The ongoing SUCCOUR trial42 may provide such information.
Evidence to Inform Guidelines Regarding the Place of GLS for Surveillance for CTRCD
Three-dimensional echocardiography, when available, is the preferred technique for monitoring LVEF and detection of CTRCD. Advantages include better accuracy in detecting LVEF below the lower limit of normal and better reproducibility. Diastolic parameters have not yet demonstrated value in predicting subsequent CTRCD.
38
Serial measurements of LVEF by MUGA are highly reproducible and have lower intra- and interobserver variability and a smaller coefficient of variability as compared with echocardiography, but have the disadvantage of radiation exposure.38
Cardiac magnetic resonance (CMR) is the reference standard in the evaluation of LV systolic function; CMR can be used when echocardiography is unreliable because of technical limitations, and when discontinuation of chemotherapy is being entertained.29,38
Cardiac Biomarkers
Cardiac troponins are the sensitive biomarkers of myocardial injury and have been studied as indicators of early anthracycline-induced cardiotoxicity. The rationale for using cardiac troponins is that a reduction in LVEF becomes manifest only after a critical amount of myocardial damage has taken place, and it is therefore a relatively insensitive marker for cardiac toxicity.
43 In a study of 703 patients with cancer, cardiac troponin I (TnI) was measured prior to chemotherapy, during the 3 days after the end of chemotherapy (early evaluation), and after 1 month (late evaluation).
44 Evaluation of LVEF was carried out before chemotherapy, and 1, 3, 6, and 12 months after the end of the treatment, and again every 6 months afterward. Three TnI release patterns were identified, each associated with a different incidence of subsequent cardiac events. Patients with TnI consistently within the normal range showed no significant reduction in LVEF and had very low incidence of cardiac events (1%) during a 3-year follow-up. Patients with isolated TnI elevation at the early evaluation, and persistent TnI elevation at both early and late evaluations had high incidence of major adverse cardiac events, mainly asymptomatic LV dysfunction and heart failure (37% and 84%, respectively).
44
Studies using modern ultrasensitive TnI assays confirmed that early increases in TnI are associated with subsequent cardiotoxicity in patients undergoing doxorubicin and trastuzumab therapy.45 Therefore, troponin can be used as a surrogate marker for subclinical myocardial toxicity. Importantly, the optimal timing of troponin measurements with regard to the specific chemotherapeutic regimen is not known.43 Some suggest baseline measurement followed by a single measurement with each cycle of chemotherapy, or after a cumulative dose ≥240 mg/m2 and before each additional 50 mg/m2.46 There is currently no data to indicate that troponin-based management of anthracycline-treated patients improves cardiac outcomes.
Although brain natriuretic peptide (BNP) and N-terminal pro-brain natriuretic peptide (NT-proBNP) are standard biomarkers used for the diagnosis of heart failure, their use as predictors of anthracycline cardiotoxicity remains to be established.29,43–45
Cardiac Biopsy
The gold standard for detection of acute doxorubicin-induced cardiotoxicity is endomyocardial biopsy (EMB).
47,48 Typical histopathological changes include vacuolization of the cytoplasm due to swelling of the sarcoplasmic reticulum and mitochondria and myofibrillar loss and/or myofibrillar disarray.
49,50 Electron microscopy demonstrates loss of myofibrils and distention of the sarcoplasmic reticulum and T-tubules. Endomyocardial biopsy can be used to grade the severity of cardiotoxicity using electron microscopy based on the percent of cells demonstrating typical changes (seven-point scale described by Billingham et al.).
48,51 Moreover, EMB demonstrates morphologic changes with low cumulative dose (e.g. 200 mg/m
2).
52 However, the correlation of biopsy scores with non-invasively assessed LVEF is poor,
52 representing the ability of the left ventricle to compensate.
5
Yet EMB is rarely used, owing to the invasive nature of the procedure and the availability of simpler sensitive markers of cardiotoxicity (e.g. troponin, longitudinal strain) and because histological evidence for cardiotoxicity can exist without evidence of cardiac dysfunction, which may not contribute to clinical decisions.
Clinical Follow-up
Identifying cardiotoxicity at a preclinical stage is paramount because an asymptomatic decrease in LVEF generally precedes clinically overt heart failure,
53 and because the potential for LV recovery increases with initiation of therapy at the preclinical stage.
6,7
Schwartz et al. were the first to show that monitoring LVEF with serial measurements of radionuclide angiocardiography can reduce the incidence of doxorubicin-induced heart failure.54 Discontinuation of doxorubicin therapy was recommended for a decrease in LVEF of ≥10% and/or to a final LVEF of ≤50% in patients with normal baseline LVEF (≥50%), and for a decrease of ≥10% and/or a final LVEF 30% in patients with baseline LVEF of 30%–50%. Doxorubicin therapy was not initiated with baseline LVEF <30%. The incidence of clinical heart failure was 2.9% versus 20.8% in patients managed with and without these criteria, respectively.54
While the importance of early identification of asymptomatic LV dysfunction is undisputed,29 there are no studies that compare the efficacy of different cardiac surveillance protocols in cancer survivors. The follow-up protocol with regard to the timing and frequency of surveillance is based on clinical judgment and patient circumstances and varies by institution. For example, the Stanford Cardiology recommendations for asymptomatic cardiac monitoring of anthracycline-treated patients include echocardiography at baseline LVEF assessment, 200 mg/m2 (or at end of 240 mg/m2 if planned total dose), 300 mg/m2, 400 mg/m2, and every 50 mg/m2 thereafter (doxorubicin equivalents).55
Another proposed schedule includes measurement of LVEF at 6 months following treatment, annually for 2 to 3 years thereafter, and then at 3- to 5-year intervals for life, with more frequent monitoring in high-risk patients.30 This protocol may not be appropriate in patients with low LVEF (30%–50%).54
The American Society of Clinical Oncology Clinical Practice Guideline recommends a baseline echocardiogram, followed by an echocardiogram between 6 and 12 months after completion of cancer-directed therapy in asymptomatic patients considered to be at increased risk of cardiac dysfunction (Table 1).29 The frequency and duration of surveillance in patients at increased risk who are asymptomatic and have no evidence of cardiac dysfunction on their 6- to 12-month post-treatment echocardiogram are not clear,6 but reassessment of cardiac function is reasonable 3–5 and 10 years after anthracycline therapy.30,31
It is recommended to discontinue doxorubicin (at least temporarily) in patients who develop clinically overt heart failure.56 The indications for withdrawal or withholding of therapy in patients with asymptomatic LVEF decline are less clear, although this strategy reduces the incidence of clinical heart failure.54 A LVEF reduction of >20% from baseline (even in the presence of normal LVEF) requires reconsideration of therapy and further frequent clinical and echocardiographic examinations (Figure 1).31 With smaller reductions in LVEF, angiotensin-converting enzyme inhibitor (ACEi) and β-blocker therapy should be initiated, with withdrawal of therapy and reevaluation after 1 month.59
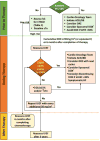 | Figure 1 Proposed Protocol for Early Detection of Subclinical LV Dysfunction and Management of Cardiotoxicity in Patients Receiving Anthracyclines. |
Of note, protocols for screening and monitoring patients for anthracycline cardiotoxicity ignore potential oncologic benefits, which vary among different tumor types, stage of the disease, and overall prognosis. There are no specific recommendations regarding continuation or discontinuation of cancer therapy in these patients. This decision, made by the oncologist, should be informed by close collaboration with a cardiologist, fully evaluating the clinical circumstances and considering the risks and benefits of continuation of therapy.29
Prevention Strategies for Anthracycline Cardiotoxicity
Dexrazoxane. Dexrazoxane (DRZ; Cardioxane®, Clinigen Healthcare Ltd, Burton on Trent, United Kingdom) is a cardioprotective agent for anthracycline-induced cardiotoxicity.57,60–64 Dexrazoxane is a bis-ketopiperazine which undergoes hydrolysis of its piperazine rings and releases a diacid diamide (an analogue of ethylene diamine-tetraacetic acid [EDTA]). Diacid diamide chelates redox-active iron before it converts O2− and H2O2 into more potent hydroxyl radicals or equally reactive iron–oxygen complexes. Dexrazoxane decreases mitochondrial iron levels, thereby preventing the formation of anthracycline-iron complexes and subsequent ROS formation.15,20,48 Another major mechanism of the protective action of DRZ is related to its ability to compete on ATP-binding sites on Top2β, producing a configuration change which prevents complex formation with anthracycline.22,65
The efficacy of dexrazoxane has been demonstrated in two randomized trials in breast cancer patients63,64 and in children with acute lymphoblastic leukemia.64,66 In a Cochrane meta-analysis, use of DRZ was associated with a marked reduction in the risk for clinical heart failure after anthracycline therapy (relative risk 0.18, 95% CI 0.1–0.32, P< 0.001).61 Dexrazoxane also attenuates the increase in troponin levels after anthracycline use.64
Because DRZ concomitantly inhibits formation of drug-induced Top2α–DNA cleavage complexes, there is a concern that DRZ may render anthracyclines less effective against cancer cells. However, a meta-analysis of randomized clinical trials found no evidence that dexrazoxane lowers doxorubicin’s anticancer effects.61
Another concern is the potential risk of increased secondary malignancy. Two randomized open studies reported a 3-fold increase in the incidence of second hematologic malignancies, particularly acute myeloid leukemia and myelodysplastic syndrome in DRZ-treated children.64,66 An increased risk of severe myelosuppression and severe infection was also reported.67
Based on these reports, the European Medicines Agency initially restricted the use of DRZ to adults with advanced or metastatic breast cancer who have received a cumulative dose of at least 300 mg/m2 doxorubicin or 540 mg/m2 epirubicin before starting DRZ.68 In the US, the Food and Drug Administration (FDA) has also approved DRZ only in patients who have received more than 300 mg/m2 of doxorubicin for metastatic breast cancer and who may benefit from continued doxorubicin treatment.69 However, long-term follow-up (5 to 10 years) of childhood acute lymphocytic leukemia patients found no differences in the incidence of secondary malignancies between dexrazoxane and placebo,70,71 suggesting that more widespread use of DRZ may be appropriate. More recently, the European Medicines Agency narrowed the contraindications for dexrazoxane to include only patients under 18 years old who are intended to receive a total cumulative dose of doxorubicin <300 mg/m2.72 The American Society of Clinical Oncology Clinical Practice Guideline also supports the use of DRZ in patients planned to receive high-dose anthracyclines (e.g. doxorubicin ≥250 mg/m2, epirubicin ≥600 mg/m2).29
Dexrazoxane is administered as a short intravenous infusion immediately prior to a bolus dose of doxorubicin at a recommended dose of 10 mg per 1 mg of doxorubicin or epirubicin.73 Because myocardial uptake of DRZ is very rapid and approaches its maximum level within 1 min, infusion just prior to the anthracycline administration is appropriate. Doxorubicin is given within 30 minutes after the completion of dexrazoxane administration. Reducing the dosage by 50% is indicated in patients with moderate to severe renal impairment (creatinine clearance values less than 40 mL/min), i.e. dexrazoxane-to-doxorubicin ratio reduced to 5:1.
Liposome-encapsulated anthracyclines. Liposomal encapsulation of anthracyclines modifies pharmacokinetics and tissue distribution without compromising tumoricidal efficacy.57 Liposomal doxorubicin is restricted to the intravascular space when capillary structure is intact with tight junctions, such as in the heart, and liposome accumulation in the myocardium is reduced. By contrast, liposomal formulations are small enough (80–90 nm) to penetrate through the more fragile fenestrated microvasculature that characterizes solid tumors, resulting in preferential accumulation in tumors and minimal release in plasma and healthy tissues, voiding the high plasma levels of free doxorubicin, which is strongly associated with cardiac toxicity.57,74,75
Two liposomal formulations have been approved for clinical use. Pegylated (polyethylene glycol embedded in the lipid layer) liposomal doxorubicin (PLD), also known generically as lipodox (Doxil®, Caelyx®), was the first FDA-approved cancer nanomedicine.76 Pegylation provides a highly hydrophilic, protective cover to liposomes, avoiding their detection by the mononuclear phagocyte system and thereby prolonging blood circulation time.74 The uncoated formulations of doxorubicin (Myocet®, a liposome-encapsulated doxorubicin citrate complex; or DaunoXome®) include citrate-increasing doxorubicin encapsulation.75,77
In the treatment of metastatic breast cancer, uncoated liposomal doxorubicin achieved a response comparable to that of conventional doxorubicin. However, the median cumulative anthracycline dose at the onset of cardiotoxicity was 785 mg/m2 and 2,220 mg/m2 for the liposomal formulation versus 570 mg/m2 and 480 mg/m2 mg/m for free drug in the studies of Harris et al.78 and Batist et al.,79 respectively. In first-line therapy for metastatic breast cancer, pegylated liposomal doxorubicin provided comparable efficacy to doxorubicin, with significantly reduced cardiotoxicity.80
When EMB was used to detect anthracycline-induced cardiac damage, PLD-treated patients had significantly lower biopsy scores compared with those of doxorubicin controls despite higher cumulative doses of anthracycline.81 A Cochrane analysis identified liposomal anthracyclines as the only formulations able to reduce the risk of anthracycline-related cardiotoxicity.82
The efficacy and cardiac safety of liposomal doxorubicin has also been documented under conditions of increased risk for cardiotoxicity such as concomitant administration of trastuzumab in breast cancer treatment.83–85 Because of cost considerations, liposomal doxorubicin is approved by the FDA in acquired immune deficiency syndrome (AIDS)-related Kaposi sarcoma, advanced/refractory ovarian cancer, multiple myeloma after failure of at least one prior therapy, and metastatic breast cancer.57,58
Prolonged administration. Anthracycline myocardial concentrations are higher after a bolus dose, and therefore cardiotoxicity is related to peak levels (Cmax).57,75 By contrast, anthracycline activity correlates with total exposure to anthracyclines, or the area under the curve (AUC). Therefore, administering anthracyclines via continuous infusion rather than as a bolus dose has been proposed to limit peak dose levels and reduce anthracycline-related cardiac effects. This approach minimally affects anthracycline AUC, and diminishes Cmax and anthracycline myocardial accumulation.75 Increasing infusion duration (>6 hours) reduced cardiotoxicity without compromising the therapeutic efficacy,57,86,87 albeit not in all studies.88 Biopsy data also support cardiac protection for 72-h and 96-h infusions.89
Clinicians may choose to use either dexrazoxane, continuous infusion (range, 6 to 96 hours),10 or liposomal formulation of doxorubicin,10,84 for prevention of cardiotoxicity in patients planned to receive high-dose anthracyclines (e.g. doxorubicin ≥250 mg/m2).3,29 Clinical experience suggests that these therapies are underused even in high-risk patients.89
Medical Therapy
Patients developing reduced LVEF during or following anthracycline treatment should be treated according to current guidelines. In patients with asymptomatic LVEF reduction, current guidelines recommend initiation of ACEi and β-blockers.
3,29,90 The expectation is that treatment with these agents will reduce neurohormonal activation, reduce adverse LV remodeling, and prevent or delay the onset of symptoms.
90 These therapies appear to be beneficial in patients with anthracycline cardiotoxicity, especially when initiated early.
6,7
Depending on the perceived benefits from the continuation of therapy, it is sometimes possible to continue treatment with the support of ACEi and β-blockade.59 Liposomal anthracycline or DRZ may also be considered in this setting.3,65
Prophylactic use of ACEi and β-blockers, prior to any documented reduction in LVEF, has been studied in all anthracycline-treated patients91 and in high-risk patients such as those planned for high-dose anthracyclines,92 those with elevated troponin,93 or concomitant trastuzumab therapy.94 Although some studies demonstrated benefit,92 this strategy remains inconclusive as some studies were neutral94–96 or demonstrated modest benefits.91,95,97 In patients with evidence of early cardiac toxicity manifesting as cardiac troponin elevation or GLS reduction without a reduction in LVEF, initiation of medical therapies may be a sensible option.3,59,69,93